|
|
Biomaterials Tutorial
Surface Plasmon Resonance (SPR)
Janet Cuy University of Washington
Engineered Biomaterials
Surface plasmon resonance (SPR) is a non-destructive analysis
technique, useful for investigating thin layers of molecules upon a
material surface. Specifically, SPR is capable of detecting changes
in refractive index (n) occurring near the surface of a metal
(within ~200nm) [1]. Refractive index refers to the speed with which
light passes through a material compared to the speed with which it passes
through air. For example, light travels more slowly through glass
than it does through air, therefore glass has a higher refractive index
than air (nglass=1.5-1.6, nair=1) [2].
The use of a metal sensing surface in SPR is critical, as this
technique capitalizes upon the fact that metals (like other electrically
conductive materials) contain electrons that behave as a continuous "sea"
of charge. This "sea" of charge can undergo charge-density
oscillations (or plasmons) at the surface of the conductor, particularly
at a surface in contact with an insulator [3, 4, 5]. Following the
"sea" analogy, imagine the behavior of seaweed washing up against a shore:
the density of seaweed (i.e., electrons) at the shore (the
interface between the "conducting" water and the "insulating" sand) varies
with the ebb and flow of the tide (the plasmon).
A simple SPR instrument set-up generally consists of a light source, a
glass prism with a high refractive index n, a thin (50 nm) metal
film placed in contact with the bottom of the prism and a photodetector
(see Figure 1) [6]. The molecular layer of interest can be coated
onto the thin metal film may be coated with a molecular layer of interest
on the side opposite the prism.
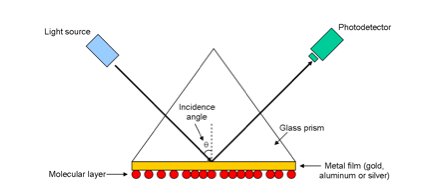
Figure 1. Basic SPR instrument configuration. Adapted from
Levesque and Paton [6].
Surface plasmon waves (SPWs) can be generated at the interface between
the conductive metal film and the insulating molecular layer by striking
the metal sensor with a particular type of light [7, 8]. At the same
time that SPWs are generated, light is also reflected off of the metal
surface. Past a specific incident angle (q in Figure 1), and only in
the presence of the highly refractive glass prism, all the energy from the
incident light wave will be transferred to the reflected light wave (total
internal reflection) [9]. However, at a very specific angle
past the point of total internal reflection (the SPR angle), a majority of
the incident light energy that would have typically been transferred to
the reflected light wave will instead interact with the generated SPWs,
resulting in a phenomenon called resonance [6, 10]. At resonance, a
minimum in reflected light intensity will be observed, and the SPR angle
can thus be determined by measuring the intensity of the reflected light
(via photodetector), and plotting it as a function of incidence angle (see
Figure 2) [10].
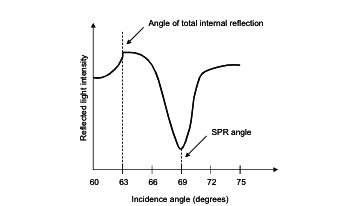
Figure 2. Example of SPR spectrum. Adapted from
Kolomenskii, et al. [11].
The SPR angle is dependent on several factors, including:
characteristics of the metal film, the incident light, and the thickness
and refractive index of the molecular layer in contact with the metal
sensing surface [10]. Consequently, spectra can be generated for a
metal surface with and without a coated molecular layer. Then, the shift
in SPR angle between the two can be quantified and used to calculate the
thickness or refractive index of the adhered molecules [12]. SPR has
proven useful in determining both growth in the thickness of a molecular
layer [13] and loss in thickness, even of a single monolayer [14].
Along with its ability to determine the thickness of coated films, SPR
has also emerged as a technology in the area of sensors (e.g.,
for the detection of physical quantities, chemicals and biologics)
[8]. Physical quantities (such as temperature and humidity) can be
deduced from changes in refractive index. Chemical sensing can use
changes in refractive index to indicate changing concentrations of
molecules adhered to the metal surface (as a result of chemical
reactions). Biosensing can also use refractive index changes to
deduce the occurrence of binding interactions (such as between antigens
and antibodies). SPR also provides the important advantage of being
able to monitor reactions in real-time, without the need to go through the
often complicated process of labeling molecules with fluorescent or
radioactive probes [15].
Like all surface analysis techniques, SPR has its limitations in terms
of sensitivity (the smallest amount of molecule detectable) [5, 8],
resolution (the smallest difference in SPR angle distinguishable) [8, 16]
and sample characteristics (geometry, thickness, etc.). However,
this technique still provides a remarkable variety of capabilities for the
characterization of reaction kinetics and thin film properties, with a
high degree of sensitivity and in real-time—all important factors for a
biomaterials scientist involved in the engineering, alteration and study
of functionalized surfaces.
References:
- Sigal GB, Mrksich M, Whitesides GM. Using surface plasmon resonance
spectroscopy to measure the association of detergents with
self-assembled monolayers of hexadecanethiolate on gold. Langmuir 1997;
13: 2749-2755.
- Foster B. Optimizing light microscopy for biological and clinical
laboratories. Dubuque: Kendall/Hunt; 1997. p. 5.
- Raether H. Surface plasma oscillations and their applications. Phys
Thin Films 1977; 9: 145-244.
- Ratner BD, Castner DG. Electron spectroscopy for chemical analysis.
In: Vickerman JC, editor. Surface analysis: The principal techniques.
New York: John Wiley&Sons, 1997.
- Garland PB. Optical evanescent wave methods for the study of
biomolecular interactions. Q Rev Biophys 1996; 29: 91-117.
- Levesque L, Paton BE. Detection of defects in multiple-layer
structures by using surface plasmon resonance. Appl Opt 1997; 36:
7199-7203.
- Caruso F, Jory MJ, Bradberry GW, Sambles JR, Furlong DN.
Acousto-optic surface-plasmon resonance measurements of thin films on
gold. J Appl Phys 1998; 83: 1023-1028.
- Homola J, Yee SS, Gauglitz G. Surface plasmon resonance sensors:
Review. Sensors Actuators B:Chem 1999; 54: 3-15.
- Fishbane PM, Gasiorowicz S, Thornton ST. Physics for scientists and
engineers. Englewood Cliffs: Prentice Hall, 1993.
- Stenberg E, Persson B, Roos H, Urbaniczky C. Quantitative
determination of surface concentration of protein with surface plasmon
resonance using radiolabeled proteins. J Colloid Interface Sci 1991;
143: 513-526.
- Kolomenskii AA, Gershon PD, Schuessler HA. Sensitivity and detection
limit of concentration and adsorption measurements by laser-induced
surface-plasmon resonance. Appl Opt 1997; 36: 6539-6547.
- de Bruijn HE, Kooyman RPH, Greve J. Determination of dielectric
permittivity and thickness of a metal layer from a surface plasmon
resonance experiment. Appl Opt 1990; 29: 1974-1978.
- Caruso F, Niikura K, Furlong DN, Okahata Y. Ultrathin multilayer
polyelectrolyte films on gold: construction and thickness determination.
Langmuir 1997; 13: 3422-3426.
- Herminghaus S, Leiderer P. Nanosecond time-resolved study of pulsed
laser ablation in the monolayer regime. Appl Phys Lett 1991; 58:
352-354.
- Geddes NJ, Martin AS, Caruso F, Urquhart RS, Furlong DN, Sambles JR
et al.Immobilisation of IgG onto gold surfaces and its
interaction with anti-IgG studied by surface plasmon resonance. J
Immunol Methods 1994; 175: 149-160.
- Tao NJ, Boussaad S, Huang WL, Arechabaleta RA, D'Agnese J. High
resolution surface plasmon resonance spectroscopy. Rev Sci Instrum 1999;
70: 4656-4660.
|
|